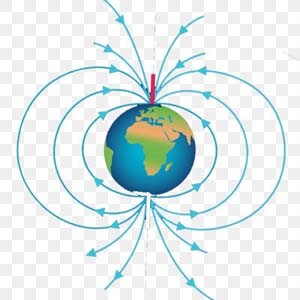
New Archeomagnetic Directional Records From Iron Age Southern Africa (ca. 425–1550 CE) and Implications for the South Atlantic Anomaly
Abstract
The paucity of Southern Hemisphere archeomagnetic data limits the resolution of paleosecular variation models. At the same time, important changes in the modern and historical field, including the recent dipole decay, appear to originate in this region. Here a new directional record from southern Africa is presented from analysis of Iron Age (ca. 425–1550 CE) archeological materials, which extends the regional secular variation curve back to the first millennium. Previous studies have identified a period of rapid directional change between 1225 and ∼1550 CE. The new data allow us to identify an earlier period of relatively rapid change between the sixth and seventh centuries CE. Implications for models of recurrent flux expulsion at the core‐mantle boundary are discussed. In addition, we identify a possible relationship of changes recorded in these African data with archeomagnetic jerks.
Plain Language Summary
Earth's dipole magnetic field is presently undergoing a rapid decay, best expressed by a deepening area of low field called the South Atlantic Anomaly (SAA). This apparent collapse of the geomagnetic field, and speculation about a future field reversal, has captured the public imagination. But we know little about the history of the SAA, limiting our ability to place current changes within a long‐term context. Here we present a new magnetic record from sites of southern Africa. The new record supports our prior inferences that the SAA is just the most recent manifestation of a recurring phenomenon in the core beneath Africa—called flux expulsion—that is having a profound impact on the expression of the geomagnetic field.
1 Introduction
The rapid decay of Earth's dipole moment over the past two centuries (Gubbins et al., 2006; Jackson et al., 2000) is also associated with rapid changes in field morphology (Hulot et al., 2002). These observations have prompted speculation that the present behavior of the geodynamo is unusual (De Santis & Qamili, 2008; Laj & Kissel, 2015; Pavón‐Carrasco & De Santis, 2016) and provide motivation for improving our knowledge of the temporal evolution of the geodynamo further back in time. In particular, efforts have focused on improving the spatial and temporal coverage of archeomagnetic and paleomagnetic records over the past two millennia. However, such data are overwhelmingly (>90%) biased toward the Northern Hemisphere, which limits the resolution of paleosecular variation (PSV) models. Additionally, the present decay in dipole moment appears to be strongly associated with both the growth of reversed flux patches at the core‐mantle boundary (CMB) in the Southern Hemisphere, as well as the expansion and deepening of the surface intensity low, which defines the South Atlantic Anomaly (SAA)—an important feature of the ionosphere. Longer‐term data from this region are crucial to understanding the current trend.
Although several recent studies have improved coverage over the African continent, for example, archeointensities (Kapper et al., 2017; Mitra et al., 2013) and directions (Donadini et al., 2015) from west African archeological sites, these locations remain at least 40° north of the CMB reversed flux patch (i.e., extrapolated to a surface location) linked to the present‐day SAA. We recently presented the first archeomagnetic data from Iron Age sites of southern Africa (∼1000–1550 CE) (Neukirch et al., 2012; Tarduno et al., 2015). These records show a sharp intensity drop (0.054 μT/yr) after ca. 1270 CE, at a rate comparable to modern field changes in the SAA, but to lower values. This was accompanied by rapid directional change of between 0.1°/yr to 0.12°/yr during the period 1225 to ∼1550 CE. The pattern of changes motivated the model proposed by Tarduno et al. (2015), whereby the recurrence of low field values reflects magnetic flux expulsion from the core, promoted by the unusual CMB composition and structure beneath southern Africa as defined by seismology (Cottaar & Lekic, 2016; Lekic et al., 2012). There are virtually no archeomagnetic data from southern Africa before ca. 1000 CE, which are needed to test the model of recurring flux expulsion episodes. We have therefore shifted focus to Botswana and Zimbabwe to sample early Iron Age structures that would extend the directional sequence back to the first millennium CE.
2 Archeological Collection
We have identified and sampled the remains of burnt daga (mud) grain bins, hut floors, and cattle enclosures (kraals) from well‐dated Early and Late Iron Age localities around the Shashe‐Limpopo confluence and surrounding plateaus (see Figure 1) in northern South Africa, Botswana and Zimbabwe. Most grain bins, hut floors, and kraals are now found as isolated patches amid brush vegetation common in southern Africa. Further archeological context can be found in Huffman (2007) (see also supporting information; Huffman, 1978; Huffman & du Piesanie, 2011; Huffman et al., 2013, 2016; Huffman & Woodborne, 2016; Main, 2002, 2008; Robinson, 1961). These localities fill gaps in previous records and extend the directional curve back to the fourth century. The material, previously unstudied, is similar to that already reported in Neukirch et al. (2012) and Tarduno et al. (2015), and dated to various episodes in the first millenium CE, with the exception of one locality (Faure Ruins, FR) which dates to the sixteenth century. All localities are dated by Accelerator Mass Spectrometry radiocarbon analysis of associated organic material, and/or the stylistic sequence of pottery (Huffman, 2007) associated with the archeological assemblage.

Figure 1
The Iron Age in southern Africa began with several phases of migration of Bantu‐speaking peoples from central and west Africa. They cultivated various grains, developed complex metal‐working technologies, and lived in villages which included grain bins, huts, and cattle enclosures (Huffman, 2007). It has been established that ritualistic burning of daga structures (Huffman, 2009) was performed in response to periods of prolonged drought (Huffman & Woodborne, 2016).
We collected fragments from four grain bin localities (Rhino Mine I, “RMEI”; Rhino Mine II, “RMEII”; Mabveni, “MB”; and Buhwa, “SL”), one hut floor locality (Manong East, “ME”), and one kraal locality (Faure Ruins, “FR”), all of which appear to have been ritualistically burnt (supporting information, Figure S1). Burnt fragments were collected from in situ material during 2014 and 2016 field seasons and orientated with Sun and Brunton compasses. In appearance, the grain bin materials consisted of a mixture of fine‐grained red clays with millimeter‐sized quartz pebble inclusions, which experienced high temperatures and rapid cooling. The kraal materials were originally a mixture of cattle dung, grass, clay, and wood, which formed a gray‐green vesicle‐rich glass with a mixture of black glass upon heating to high temperatures. The RME grain bins were exposed by iron ore mining operations in the vicinity, but apparently not disturbed from their original positions. Because the area is rich in iron ore, we conducted a field survey (see supporting information) to map possible magnetic anomalies using a Grad601 high‐resolution fluxgate gradiometer (Bartington Instruments). The observed field anomaly around the localities was sufficiently low (<200 nT) to ensure that grain bin materials acquired a directional signal representative of the geomagnetic field during cooling after firing (supporting information, Figure S4).
3 Magnetic Mineralogy
Rock magnetic experiments were performed at the University of Rochester to characterize magnetic mineralogy. Low‐field susceptibility versus temperature measurements were performed on bulk powdered material in air using a KLY4‐CS Kappabridge (AGICO). The results indicate stability upon heating, with Curie temperatures in the 560 to 600°C range. Magnetic hysteresis and first‐order reversal curves (Pike et al., 1999) were measured using an alternating gradient force magnetometer (MicroMag 2900, Princeton Measurements Corporation). These indicate the presence of noninteracting single domain/pseudo‐single domain grains, consistent with magnetite/titanomagnetite carriers (see Figures 2, S2, and S3; Day et al., 1977; Dunlop, 2002), with some high‐coercivity background phase in most samples, likely pigmentary hematite. For one locality, ME, hysteresis loops are slightly wasp‐waisted, which suggests the presence of two remanence‐carrying fractions of different coercivities. Magnetic susceptibility data indicate the dominance of a magnetite or near‐magnetite carrier. Various degrees of reproducibility were observed in cooling curves, indicative of changes in domain state or composition. The latter suggest the presence of minor magnetic phases (e.g., maghemite and hematite).

Figure 2
4 Archeomagnetic Directions
Cube specimens (∼1 cm3) were prepared for laboratory measurements using a bronze rock saw (UK‐650, ASC Scientific), which was kept cool by the application of a moist sponge to the blade during operation (Figure S1). After cutting and drying, specimens were stored for several days in the magnetically shielded room (ambient field <200 nT) at the University of Rochester. Measurements were then performed with a 755R DC‐SQUID magnetometer (2G Enterprises) with high‐resolution sensing coils. Alternating field (AF) demagnetizations were conducted in 5 mT steps from 5 mT to 40 mT, and thereafter in 10 mT steps to 100 mT using a SI‐4 AF demagnetizer (Sapphire Instruments). Thermal demagnetizations were also performed on specimens from each fragment in 25°C steps from 150°C to 625°C using a TD48‐SC thermal demagnetizing oven (ASC Scientific).
Orthogonal vector plots display stable single‐component magnetizations, which trend to the origin during both AF and thermal treatments (Figures 3a–3f. For most specimens, approximately 50–70% of natural remanent magnetization (NRM) is lost between the 10 and 50 mT demagnetization steps. After the 100 mT AF treatment, ∼20% of the original NRM is retained, suggesting the presence of a high‐coercivity hematite phase. Directions obtained by principal component analysis (Kirschvink, 1980) in a range between 15 mT and 100 mT (depending on specimen) display maximum angle of deviation (MAD) values generally less than 3°. Specimen directions were then used to calculate the Fisher mean directions and 95% confidence interval (Fisher, 1953). Prior thermal remanent magnetization acquisition experiments show no evidence for thermal remanent magnetization anisotropy (Tarduno et al., 2015) in these materials.

Figure 3
Most of our samples showed acceptable MAD values (more than half of MAD values are less than 2.3°), but occasionally, MAD values are much greater (5–21°). These MAD values, as well as dispersions associated with some mean directions, are higher than seen in studies of archeomagnetic materials obtained elsewhere (e.g., Europe) and most likely reflect the heterogeneous nature of the material and postfiring disturbance and alteration (weathering). Accordingly, we adopted a stringent procedure to identify and exclude outliers. The procedure is similar to that used by Tema and Kondopoulou (2011) and Pavón–Carrasco et al. (2010). Briefly, we reject outlier directions which display angular distances more than 3 × α95 from the Fisher mean direction of each locality. The mean and α95 is then recomputed without the outlier direction(s). Using this procedure, we excluded between zero (RME2) and six (ME) specimens per locality. More information about this procedure can be found in the supporting information (Figure S6), and all individual specimen data may be accessed on the MagIC database. Because we believe that each burnt structure at a locality is of the same age, each fragment has approximately the same number of measured samples at a locality and there is scatter at the specimen level (detected by our outlier analysis, see Tables S2–S7); specimen directions were grouped at the locality level (Table 1). An exception to this is the splitting of RME into two occupation ages (Table 1 and supporting information). Values of α95 were generally around ∼5° (see Table S1), and only one site, FR, exhibited an α95 value greater than 5°.
Locality | Age CE | N'/N | D (deg) | I (deg) | k | α95 (deg) |
---|---|---|---|---|---|---|
Faure Ruins (FR) | 1500–1610 | 11/12 | 352.4 | −35.8 | 39 | 7.4 |
Rhino Mine I (RME) | 750–800 | 17/25 | 21.7 | −22.4 | 73 | 4.2 |
Mabveni (MB) | 675–700 | 17/18 | 11.8 | −16.7 | 76 | 4.1 |
Manong East (ME) | 590–636 | 26/32 | 10.3 | −23.7 | 101 | 2.8 |
Rhino Mine II (RME) | 550–570 | 13/13 | 3.0 | −29.9 | 80 | 4.7 |
Buhwa (SL) | 400–450 | 19/36 | 2.7 | −38.3 | 60 | 4.4 |
- Note. N'/N, number of specimens from locality used in analysis/number of specimens measured; D (deg) mean declination; I (deg) mean inclination; k, an estimate of the dispersion of the population of directions; α95, confidence limit for n number of directions. See main text and Tables S1–S8 for more information.
Finally, we reduced locality mean directions to the location of Mapungubwe (22.212°S, 29.387°E), the capital of a precolonial Iron Age Kingdom. Mapungubwe is an important archeological site geographically located at the approximate center of our site distribution, making it a convenient choice for the reduction. Mean directions were reduced to these coordinates using virtual geomagnetic poles, VGPs (Shuey et al., 1971). The magnitude of the correction is between 0.1 and 5°. To estimate the error due to VGP reduction, we compared the International Geomagnetic Reference Field (IGRF) value for each locality for the year 2010, reduced to the location of Mapungubwe, with the known value (Dec, 346.98°; Inc, −60.56°). The angular difference between directions is less than 2°, which is similar to the result obtained for archeomagnetic data from western Europe (Gallet et al., 2002) using an identical procedure. However, we caution that gradients may have been greater (and greater than in Europe) in the past and the VGP reduction procedure we have employed should only be taken as a guide. The progression of unreduced directions is shown in Figure S5. Table 1 shows the final site mean directions and statistics. A complete list of individual specimen directions may be found in the supporting information.
In Figure 4 we plot our records on an equal‐area stereonet, combined with earlier data from Neukirch et al. (2012) and Tarduno et al. (2015). The new results define a coherent loop in the archeomagnetic curve for southern Africa between ca. 425 and ca. 1370 CE. In other words, the earliest site (SL, 400–450 CE) shows directions that are statistically indistinguishable from those more than 900 years later (AD 160, 1317–1415 CE).

Figure 4
Here we divide this new archeomagnetic directional path into five arc segments, separated by cusps (Figure 4 and Table S8). While this division is somewhat subjective, we feel that our choice accurately reflects the important trends defined by the data. Other divisions that are sensitive to the need to average over intervals long enough, such that uncertainties in age and direction do not obscure the major trends (>100 yr), yield similar rates. Previous work identified a period of relatively rapid directional change between 1225 and ca. 1550 CE (>0.1 °/yr). In our new data, we see an earlier period of relatively rapid change between ca. 400–450 and ca. 550–570 CE, and again between 550–570 CE and 750–800 CE, during which the rate of change was approximately 0.1 °/yr. This is more rapid than the modern rate of change in the Limpopo region of 0.07 °/yr (here estimated from the IGRF direction and the predicted direction for 1840 from the CALS3k.4, Korte et al., 2009, model).
5 Discussion
Rapid directional changes between 1225 and ∼1550 CE are accompanied by intensity values that are lower than the present‐day regional low, which is associated with Southern Hemisphere reversed flux patches at the CMB (Tarduno et al., 2015; Terra–Nova et al., 2017). This is suggestive of repeated episodes of flux expulsion associated with the African Large Low Shear Velocity Province (LLSVP) influencing core flow (driving the magnetic Reynolds number toward unity) leading to reversed flux (Tarduno et al., 2015). The longevity of the LLSVP suggests that flux expulsion could be a recurring feature in this region. Therefore, similar episodes of rapid directional change are expected further back in time. While not as rapid as the changes in the fifteenth century, the directional variations identified between 400–450 CE and 750–800 CE may indicate a similar flux expulsion episode. However, archeointensity values from this period are needed for further clarity.
It is interesting to compare our Iron Age directional records with PSV models (Figures 5a–5e). There is disagreement between our data and the predictions of CALS3k.4 (Korte & Constable, 2011), particularly in inclination in the First Millennium CE, whereas model PFM9k (Nilsson et al., 2014) does not represent the directional loop seen in the data (see also supporting information, Figure S7). In contrast, some other models weighted toward archeomagnetic data predict a directional loop similar to that seen in the data but at somewhat different times and predicted declinations (ARCH3k.1(MAST), Korte et al., 2009; A_FM, Licht et al., 2013; and SHA_DIF_14K, Pavón–Carrasco et al., 2014). The general agreement of data and predictions from A_FM, ARCH3k.1(MAST) and SHA_DIF_14 K is surprising; these models are strongly biased toward Northern Hemisphere data and their accuracy for Southern Hemisphere locations is expected to be limited.

Figure 5
Gallet et al. (2003) originally identified phenomena known as “archeomagnetic jerks” in secular variation records from western Europe, showing repeated periods of sharp directional variation which coincide with strong hemispheric field asymmetry, defined in terms of the maximum ratio of the quadrupolar to the dipolar energies at the Earth's surface, 〈Q〉/〈D〉. Gallet et al. (2009) found a correlation between the regional signature of these episodes and periods of strong relative quadrupole moment at ∼200 CE, ∼800 CE, and ∼1400 CE, which led them to believe that the mechanism behind archeomagnetic jerks was of global origin. They suggest that these features were caused by transient hemispherical asymmetry of flux patches at the CMB, which Dumberry and Finlay (2007) speculated were due to a long‐term influence of the lowermost mantle on thermal coupling.
In Figure 5f we show the ratio of quadrupole 〈Q〉 to dipole 〈D〉 energies for three models (i.e., ARCH3k.1(MAST), A_FM, and SHA_DIF_14K), which best approximate the archeomagnetic loop seen in our new data, together with the times of the archeomagnetic jerks identified by Gallet et al. (2009). Pavón–Carrasco et al. (2014) also discuss quadrupole/dipole energies versus time. There is excellent agreement between the rapid changes in the southern African data between ca. 1225 and ca. 1550 CE and the archeomagnetic jerk proposed by Gallet et al. (2009) at ca. 1400 CE. The rapid changes are also very clear in the ARCH3k.1(MAST) and SHA_DIF_14 K models, but not well expressed in the A_FM model.
Rapid changes in the southern African data, and the ARCH3k.1(MAST), A_FM, and SHA_DIF_14 K models occur close to the archeomagnetic jerk proposed at ca. 800 CE. However, the southern African record appears to be offset slightly (100 years or less) from the proposed archeomagnetic jerk. The offset might reflect limited resolution of our data near 800 CE or inaccuracies in PSV models.
Irrespective of differences in between the models and data uncertainties, the approximate agreement between the times of rapid changes reflected in PSV models weighted toward archeomagnetic data and our new records appears to corroborate the interpretation (Gallet et al., 2009) that global features are present in the European data. The consistency of regional data from such different contexts adds weight to the suggestion that the underlying mechanism must be of global origin. We speculate that the phenomenon of archeomagnetic jerks is consistent with repeated flux expulsion at the CMB, ultimately caused by core flow influenced by the African LLSVP.
We note that the episode at ca. 1400 CE appears to be a particularly robust feature and is also reported in sedimentary directions from the coastal lake of Eilandvlei, on the south coast of South Africa (Wündsch et al., 2016). Directional measurements of volcanic sequences from Marion Island (Amerigian et al., 1974), southeast of South Africa, show that the present high rates of directional change persisted during the past 500 kyr, further supporting the suggestion that repeated flux expulsion, and the SAA, are long‐term features of the field.
6 Conclusion
To understand present‐day and historical changes in the geomagnetic field, and to further evaluate the hypothesis of periodic flux expulsion, we presented archeomagnetic data from southern Africa which extend the existing directional curve back to the first millennium. The data record a second earlier episode of rapid directional change, and define a loop, which is absent from CALS3k.4. The coherence with archeomagnetic field models and other regional data supports a global origin for these phenomena, which is most likely periodic Southern Hemisphere flux expulsion. Further modeling, as well as archeointensity analyses from these materials, will help to shed light on the timing of these episodes and to further constrain the dynamics of core processes. We briefly note that the improved resolution offered by our record raises the possibility of archeomagnetic dating in this region.
Acknowledgments
This research was supported by National Science Foundation grant EAR‐1448227 to J. A. Tarduno. Excavation was conducted under permits from the South African Heritage Resources Agency. Several students helped with field collection: Susanna Chhibber, Sebastian Fearn, Shiri Goldman‐Nedergaard, Timothy O'Brien, and Hannah Tompkins. Monika Korte kindly provided spherical harmonic coefficients for the CALS3k.4 and ARCH3k.1 models. We thank the anonymous reviewers for their helpful comments. J. A. T., T. H., M. W., P. C. T., and M. M. conducted field collections, R. D. C. and V. J. H. conducted and supervised sample preparation and laboratory measurements and analyzed magnetic data together with J. A. T., V. J. H. and R.K.B. analyzed geomagnetic model predictions, and V. J. H. recognized the correlation with geomagnetic jerks. V. J. H. wrote the first draft of the manuscript and received subsequent contributions from the coauthors. J. A. T. conceived the project with T. H. and M. W. and supervised the overall investigation. New data presented here are available in the Earthref (MagIC) database, http://earthref.org/MagIC/16376.